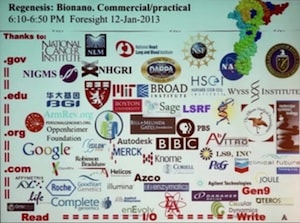
A select set of videos from the 2013 Foresight Technical Conference: Illuminating Atomic Precision, held January 11-13, 2013 in Palo Alto, have been made available on vimeo. Videos have been posted of those presentations for which the speakers have consented. Other presentations contained confidential information and will not be posted.
The 1st speaker at the Commercial Scale Devices session was George M. Church. His talk was titled “Regenesis: Bionano ” biography and abstracts, video – video length 51:19.
Prof. Church began with the immense drop in the cost of DNA sequencing from the production of the draft human genome sequence in 2000 at a cost of $3 billion to the early 2013 cost of $2000 to $4000 per genome. With whole genome sequencing becoming inexpensive, the question arises where are you going to store all of the data? Prof. Church’s answer is that DNA itself is a pretty good place to store that data, and other types of data as well. In fact, he encoded his book co-authored with Ed Regis Regenesis: How Synthetic Biology Will Reinvent Nature and Ourselves, into a 5.27 megabit bitstream that was written with DNA synthesis technologies and read with DNA sequencing technology, requiring about a picogram of DNA, implying about a MByte/femtoliter (“Next-Generation Digital Information Storage in DNA“). Church notes that DNA as old as 800,000 years has been sequenced, and that he personally made 70 billion copies of his book.
Turning to the topic of DNA nanostructures, Prof. Church began with the development by Shawn Douglas, at the time a graduate student he shared with William Shih’s group, of a computer-aided design tool for DNA nanostructures called cadnano. One use of this tool, again done in collaboration, was to make long rods of DNA that were used as fluorescent barcodes with 42 nm spacing, in which the color and intensity of each element of the barcode varies with transient binding of short oligonucleotides. The barcodes can thus be used to identify cells or molecules at a disease site, for example. In another collaboration begun in Church’s group by Shawn Douglas and Ido Bachelet designed a nanorobot. This is a hybrid nanostructure that not only incorporates a DNA scaffolding, but also includes DNA aptamers that are active logic gates (see this Nanodot post from three years ago and this recent follow up). One strand of a DNA lock can bind to cell surface structures diagnostic of, for example, a cancer cell, and in response cause the DNA to open the DNA scaffold and release drug or antibody payloads. Only if the DNA locks on both sides of the structure finds suitable ligands on the surface o the cell, do both locks release and open the structure. So the nanorobot is basically doing an “AND” logic operation to determine if it should open and attack the cell. Other recognition sites and logic operations are possible. Because these nanorobots have sensors, logic, and actuators, they meet the minimum qualifications to be called “nanorobots”. These potential applications show that DNA origami has traveled a long way from “smiley faces”>
The third topic Prof. Church addressed is the application of nanotechnology to DNA sequencing. Of 44 projects, 9 have already had substantial commercial impact. An important requirement in DNA sequencing is to look at single molecules one at a time, but to do in as highly parallel a manner as possible. An important feature of many of these technologies is to connect microfabrication with single molecules of DNA. State-of-the-art 10-nm microfabrication is not required; crude microfabrication with 300 nm spots and 600 nm spacing suffices. Because the size of a single DNA nanostructure of a rolling-circle-amplified DNA molecule is comparable to the size of the microfabricated spot, you get exactly one molecule per spot. Each spot is uniquely addressable over many centimeters squared.
Another application of single molecule DNA sequencing is nanopores, of which there are several approaches. You can detect polymers going through a nanopore, and thus affecting the stream of ions going through the nanopore. Or the nucleotides can be clipped of with an exonuclease so that so that individual A’s, C’s, G’s, and T’s can go through one at a time. Or the nucleotides can be chemically modified and are then called “nanotags”. The nanopores are proteins obtained from biology, but heavily engineered. Further, they want to fuse the pore proteins with a DNA polymerase that will step the DNA though the pore like a stepper motor. They are not limited to simple fusions of the two proteins, but rather can cross-link them post-synthetically and add non-standard amino acids not found in biology to get very specific cross-links and make very interesting nanomachines that can be made in bulk and interfaced with CMOS electronics.
These advances in genome sequencing are opening the door to soon getting everyone’s genome sequenced, leading Prof. Church to his fourth topic: genome engineering—specifically one near-term approach using zinc fingers for the long-term control of HIV. The story begins in 2007 with one individual unfortunate enough to have both leukemia and AIDS. A standard treatment for leukemia is to get histocompatibility-matched hematopoietic stem cells from a donor. Coincidentally the matching donor who was identified for him also carried a very rare mutation that was deleted for both copies of the CCR5 gene, which was already known as a possible co-receptor along with CD4 for the HIV virus particle. As a result, his stem cell transfer in 2007 cured both his leukemia and his AIDs, and as of 2013 he was still cured. This result inspired an effort to use zinc finger nucleases as a therapy in other AIDS patients to target their T cells to specifically remove both copies of their CCR5 gene so that their T cells cannot be infected with HIV. Currently this therapy is looking promising in phase 2 clinical trials.
The zinc finger technology has been around for two decades and involves DNA-binding proteins that have about 3-base specificities per alpha-helix so that three or four on each side of a DNA sequence can be coupled together to recognize about 20 base-pairs of genomic DNA. The dimer has a nuclease in the middle that allows you to either mess up the gene or bring in some DNA to precisely engineer a human genome. For two decades zinc fingers were the most easily engineered of the specific DNA binding proteins. Last year we and others introduced TAL-effectors (TALEs), from a bacterial plant pathogen, that is much easier to engineer for DNA specificity, but which takes 2 kilobases of mRNA for TAL to change one base pair in the human genome. So just last week we published a new alternative—CRISPR, which is a protein-RNA hybrid where the heavy lifting of finding the few human base pairs to change in a genome of six billion base pairs is done by a short RNA. With CRISPR we have gone from making one change in the human genome being very challenging to being able to print tens of thousands. CRISPR is being used to change skin cells from a volunteer into induced pluripotent stem cells, which can become any cell in the body. An exciting possibility presented by CRISPR is the possibility of multiplexing—changing not just one gene but many genes at once. In one example a bacterium used in gene studied was recoded to give three new functions: genetic isolation, codon reassignment so that many non-standard amino acids could be incorporated into proteins, and multi-virus resistance. By taking advantage of the redundancy of the genetic code, up to 13 codons can be changed, genome-wide, without harming the ability to use the organism.
To see how profoundly the genome can be altered, Prof Church and his group have identified the minimal set of genes necessary for robust growth,even replicating faster (10 minute doubling time) than the standard bacterium used in genetic engineering. The list of components they think are needed for replication and protein synthesis comprises 151 genes (RNAs and proteins) and only 131,000 base pairs. They are in the process of synthesizing each of those components in vitro so that eventually they will be able to reconstitute an entirely synthetic organism. Such organisms could be resistant to not only all viruses but also all enzymes. One of their purposes is to study biopolymers made from monomers that are the mirror images of the monomers found in nature. Proteins and nucleic acids made with mirror monomers could have radically new properties but still be predictable enough in their structures to allow protein engineering. They are currently trying to make a mirror image polymer of one of the smallest DNA polymerases capable of PCR amplification to make mirror image PCR possible, and also to make ribosomes capable of incorporating mirror image amino acids.
They can now incorporate new, non-standard amino acids in genomically recoded organisms (GROs) in which the genetic code has been changed. This process is efficient because the new codons are not competing with the standard codons as in normal cells. In collaboration with Peter Schultz and others they are experimenting with new amino acid chemistries that are orthogonal to the standard chemistries. For example, azides can react with alkynes to make triazoles, or ketones can react with hydrazides, or they can react with phosphine or hydroxylamine. None of these chemistries are particularly cross-reactive with biochemistries already in the cell, but they are highly reactive with each other, so it allows you to do cross-linking and other things in a very specific manner. This technology has immediate commercial components as well. There are ten companies that do in vitro protein synthesis and at least one company uses non-standard amino acids to stabilize human protein pharmaceuticals.
The final topic that Prof. Church addressed is a brain activity map, in which the interaction between polymerases and ion channels is very important in a way that is quite different from its application to DNA sequencing, discussed above. Increasing our ability to do input and output to the brain has a large umber of health and practical implications, particularly with respect to neurodegenerative diseases. One of the promising pathways is not to use electrodes, but instead to use light, both for input and output, using a variety of molecules that can be harvested from bacteria and a number of invertebrates. Currently we can simultaneously measure about 100 neurons; we would like to be able to do billions. The problem with embedding too much electronics is the consumption of a lot of energy per neuron. Church’s lab is currently working on using DNA polymerases, which are about a billion-fold more energy efficient than electronics, to measure neuronal activity, for example by the effect of calcium ion concentration on misincorporation into DNA using a known template. Deep sequencing thus provides an indirect readout of calcium ion concentration in the vicinity of the polymerase, and thus yields information about neuronal activity.
—James Lewis, PhD