Summary
In this session, Michael Levin, director of the Tufts Center for Regenerative and Developmental Biology, introduced the field of bioelectricity, and the impressive regenerative potential it harbors. Michael showcased techniques that enable regrowth of limbs and formation of different organs using bioelectricity and cocktails of repurposed ion channel drugs. And he also gave an answer to the fundamental question of where does shape come from and how we could possibly program it in any way we want.
Presenters
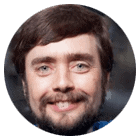
Michael Levin, Tufts Center for Regenerative and Developmental Biology
ichael Levin, Distinguished Professor in the Biology department and Vannevar Bush Chair, serves as director of the Tufts Center for Regenerative and Developmental Biology. Recent honors include the Scientist of Vision award and the Distinguished Scholar Award. His groupās focus is on understanding the biophysical mechanisms that implement ā¦
Presentation: Michael Levin
- Transformative medicine will address aging and many other things, which is why regenerative medicine is so important.
- If I had to boil down the talk to one sentence, this would be it ā all of your body cells and tissues make decisions about structure and function, and we now have the techniques to read the information processing that goes into those decisions and actually modify it rationally.
- This is our 5 legged frog and this is a reminder that you will see all kinds of weird creatures today and these are not photoshopped, these are actual real animals that we produced during the testing of our theories of where shape comes from and how to control it.
- I want to highlight what we donāt know despite our amazing advances in molecular biology.
- What we would like to have is something we call the anatomical compiler. The idea is that someday you should be able to sit down at a computer and draw the animal or plant that you would like to have. Not try to build it bottom up out of distribution of pathways, but just say that you want a three headed flatworm with a shape like this. And the compiler will compile that anatomical description into a set of stimuli that have to be produced to create it. Thatās the endgame for this field ā total and complete control of morphology. It might be a liver, a heart, a whole organism, or something that never existed before ā it shouldnāt matter.
- The critical thing is that if we could do this, we would immediately have all the answers to birth defects, regeneration of lost or damaged organs, defects from cancer, aging, all of these challenges to healthy normal morphology of a body.
- This is ultimately a problem of information processing. The question is why donāt we have this yet?
- Because there are fundamentally gaps in our understanding. We donāt have a clue if chimeric embryos of axolotl larva and frog larva will have legs. We have both genomes, but we cannot explain these basic fundamental questions just based on genomes. And that really holds back regenerative medicine.
- So letās ask the fundamental question: where does shape come from? Here you see the cross-section of the human torso. Look at this incredible arrangement, organs are all in the right place, orientations, sizes, positions relative to each other. Where does all this come from? Genome produces the cellular hardware that is needed to create this structure, it gives you all the protein sequences, but it doesnāt directly say anything about this kind of order. We really need to understand how cell groups know what to make and when to stop. All these cells are active agents that do various things, and the result of their activities self-assemble into this amazing organism.
- As people working on regenerative medicine we need to ask ourselves how to convince those cells to rebuild again. And as engineers we can ask what else we can do with them, can we build something completely different?
- Important thing is that single cells are incredibly competent. You can see this one cell hunting, it handles all of its physiological and anatomical and behavioral needs all in one cell ā without any brain or nervous system, no stem cells or cell to cell communication. Itās just one cell doing everything it needs.
- When cells get together, they actually scale up this ability and work on much bigger goals ā production of complex and variant anatomy. But itās not enough to have the building blocks, you need to know how they go together, otherwise you end up with a teratoma.
- The mainstream paradigm thinks about the feed-forward emergent process. The difficulty with this paradigm for regenerative medicine is that if you want to make repairs to the anatomy, you have to somehow invert this chain to somehow figure out which genes you have to edit and how in order to have the complex anatomical changes. And this inversion is extremely difficult, fundamentally intractable. This is basically what holds regenerative medicine back, we simply donāt know how to figure out what changes to make at the lowest levels to give you the desired effects on the system level.
- The field is really good at figuring out which genes and proteins control each other, and how those pathways operate. But what we really want to understand is where do these different shapes come from and what do we do to alter them rationally.
- Itās not only about creating a complex body during embryogenesis, itās also about the amazing ability of the cellular collectives to repair over time. Axolotls during their lifespan regenerate many of their organs. No matter where you amputate during their lifetime, they will do exactly the correct amount of growth and patterning to have an indistinguishable replacement in the end.
- Whatās remarkable about this is that not only does it create the right tissue, it also stops at the right time. Until a correct replacement is formed, there are many changes in the gene expression and rapid cell proliferation, but once it is formed, it all grinds to halt. How does it know when the correct replacement has formed? Thereās an amazing degree of plasticity and adaptive problem solving.
- Axolotls are not the only organisms to regenerate.
- This should be a motivational and inspirational animal for everybody interested in healthy lifespans. These are complex animals with true brain and bilateral symmetry, not like earthworms. They have the ability of regeneration ā you can cut them into pieces, the record is something like 276 pieces, and every piece will regenerate exactly whatās missing and give you a perfect new little worm. Not only that, they are smart, they can learn, and they are immortal ā there is no such thing as an old planarian. Individual cells fall off, regenerate whatās missing. What this creature is telling us is that it is possible to be an immortal highly regenerative creature that also has intelligence.
- One of the really important aspects is the idea of problem solving by the collective intelligence of cells. Here is an example ā this is a tadpole face, which needs to become a frog. It needs to rearrange the face in order to become a frog. It used to be thought that genetics give you a sort of hardwired set of movements. What we did is called Picasso tadpole, where everything is in the wrong place. When this happened, they still in the end formed largely normal frogs. Why? Because all of the different components move around in different paths, until they get to a normal frog shape. That is remarkable, because it means that what genetics actually gives you is a machine that is able to minimize error, that it has some internal representation of what a correct frog face looks like. If we had swarm robots that could do this, it would be a remarkable example of collective intelligence.Ā
- This collective intelligence and adaptive problem solving is something we need to solve in addition to the mechanistic pathways in order to really solve anatomy and to really solve regenerative medicine.
- What we would like to do is to really understand this at an algorithmic level.
- Computer science offers us an interesting analogy. Earlier to program, you had to physically move the wires around, physically change the structure of the machine. What we then realized is that if the hardware is good enough and reprogrammable, you donāt need to physically change the hardware, but interact by means of inputs or experiences and take advantage of the software inside.
- All of modern biology are single molecule approaches, weāre rewiring pathways, genome editing, weāre getting more and more specific at the low level of the hardware. That is important, but it needs to be coupled with what the software looks like, and how much can we get with stimuli instead of rewiring be it genetically or otherwise.
- The framework that we work in looks more like this. There is the feed-forward component, but there are also very important feedback loops, where any challenge to the body (traumatic injury, aging, pathogens,ā¦) triggers a set of feedback loops both at the level of physics and genetics, that serves to get back to the target correct morphology ā a homeostatic cycle.
- There are two unusual things in this model. The first is that the set point for this homeostatic process is not a simple number or scale like a temperature, ph, hunger, but itās actually a much more complex structure thatās a coarse graining of the anatomy. The second part is that this is a process that has a goal ā a set point. It is a system that is fundamentally able to expend energy and effort to get back to the correct region of state space by activating all kinds of cellular behaviors ā to minimize error.
- If we make this hypothesis, it has a few strong predictions. You ought to be able to find the medium in which the target morphology is encoding ā the set point has to be recorded somewhere. And then it means that we can alter that set point, and ask the machine to build something different without having to rewire, like with a thermostat when the same system maintains a different temperature ā there is a distinction between the data and execution.
- This is what weāve been for a few years now. Asking ourselves: āCan we find the representation of target morphology and exploit it for regenerative medicine?ā
- And I think we found an important chunk of it.
- In order to tell you how that target morphology is encoded, I have to introduce you to developmental bioelectricity.
- All cells in a body are sitting in a morphogenic field of information that comes in all kinds of different media ā chemical gradients, extracellular matrix, tensions and stresses and so on. Bioelectricity is also one of those media.
- What is bioelectricity? Letās think about what happens in the brain. Networks of neurons have ion channels, they create voltage potentials, and they can pass their electrical states to these little electrical synapses known as gap junctions.Ā
- Networks of these cells have this interesting property that they can execute physiological information processing, that we associate with memory, behavior, gold directed activity, preferences, etc. Neuroscientists think that if we understand the encoding, the neural electrical code, we can look at the activity and extract semantic information. We would know what the meaning is to the animal, the content of that memory,.. So people are working on and have had some success with all kinds of methods trying to decode the information from the brain.
- This property in the brain mustāve had some simple evolutionary precursor, and it did. The evolutionary precursor is that all cells do this, not just neurons. All cells have ion channels, most cells have gap junctions to their neighbours, and this scheme of putting electrically active subunits into networks is about as old as bacterial biofilms ā extremely ancient evolutionary mechanism.
- The electrical activity in the brain is designed to activate muscles to move the body to solve the problems in 3D space, developmental bioelectricity processes information to move the body through morphospace ā to change and control morphogenesis. And we can decode it the same way we do with neurons and the brain.
- My lab has developed some tools to do this. First is voltage dye that allows for real time reading of all the electrical conversations that these cells have. Then also a lot of computational modelling.
- And then functional techniques, where you can go in and change the electrical information processing in the tissue. We do not use electrodes, apply electric fields, no electromagnetics. This is purely molecular physiology that targets the native processes by which these cells are communicating electrically. There are ways to control the topology of the network ā which cells talk to which other cells. We can open and close gap junctions and so on, and the same for ion channels ā we can go in and set the states using either optogenics or drugs or mutations that change the channel properties. So basically taking all the tools of neuroscience and using them for morphogenic decision making outside of the nervous system.
- This is an example of how some of these endogenous patterns look like. These bioelectrical gradients are a prepattern, a subtle scaffold of the direct downstream gene expression and anatomy. And if we go in and change the distribution of voltages, you will change the gene expression and the anatomy.
- Using bioelectric map you can see cells with aberrant electrical potential, you can see that they are dissociating from the electrical network, they are basically reverting to unicellular state, and they are going to treat the rest of the animal as external environment ā and thatās what a metastasis is, failure to participate in embryogenesis, because the first thing that oncogenes do is they uncouple the cells from the electrical network that normally tells every cell what they should be doing.
- The reason why this is important is that when you inject things that cause tumors, you can prevent tumor genesis, even though the oncogene is very strongly expressed.
- Going beyond single cell properties of conversion to cancer, we can look at morphogenesis. We can look at a tadpole and take a region of a gut, and inject some ion channels that will set a voltage state that is normally associated with making an eye. These cells will then in fact build an eye out of cells that were supposed to be the gut. And you can make these eyes out of tails, spinal chords, whatever. They have all the correct layers, retina, optic nerve, all of that, and they also instruct their neighbors. The blue cells you see on the image are the ones with changed voltage, but they also recruited a whole bunch of other cells which we never touched directly. So we can use this technique to induce eyes, brains, hearts, and many other things, but there are also many things that we donāt know how to make yet.
- The point is that these bioelectrical gradients are instructive for anatomy. They control at the large scale. And we donāt have to micromanage this process. What we basically found is an instructive hook into subroutines that says: āMake an eye hereā ā we donāt have to go in and provide information about how to make it, about all the layers, cell differentiation cascades, all the genes, etc. That would be incredibly difficult, we are nowhere near that as a field. But what we can do is to find the native modular control structure in the bioelectrical layer that determines which organs get placed in which regions. And the cells will take it from there once you instruct them.
- Letās look at planaria ā when you chop off head and tail, you get this middle fragment. So it has to build it again, and it has this interesting voltage gradient that says one head (depolarized region) and one tail (polarized region).
- And if we go in and artificially manipulate the voltage in the polarized region (tail) into depolarized (head), we will have a two headed animal. And these are perfectly viable, you can have a two head animal, no headed animal.
- All weāve done is activate a ābuild a headā subroutine in a particular region without knowing too much about how we go about building a head in the first place. Cells will turn on all the genes they need once they receive this high level instruction.
- Not only can you build ectopic heads of the same animal, you can build heads appropriate to other species. If we take this planarian with a triangular head, chop off the head, perturb the electrical network, and you can get flatheads like P. Felina, round heads like S. Mediterranea, or your normal triangular heads. Itās not only the head shape but also the brain shape inside as well. Because the space of possible outcomes of this electrical space contains attractors that correspond to other species that evolution has found in the past. You can dial in those without any changes to the genomics.
- And in fact you can access regions of that state space that evolution doesnāt use because they are not viable. You can take flat planarian and turn it into these weird spiky forms, hat like forms, and many more. The point is that you can have control over the large scale anatomy using the same genome, because what the genome builds is cellular collectives that use electricity to store their set points, their target morphologies. And this is a very familiar story for neuroscientists, because thatās exactly how brains work.
- Getting to the applied regenerative medicine angle. Unlike salamanders, frogs do not regenerate their legs. But weāve taken froglets and weāve used this information to design a cocktail of drugs that change the electrical state of the wound ā normally you amputate the leg and there is nothing after 45 days. WIth our cocktail, after 1 day exposure, it kickstarts the whole cascade ā pro-regenerative genes become turned on (msx1 in this case), and then immediately the limb starts to grow. By 45 days youāve got toes and toenails, eventually the whole leg is both touch sensitive and motile.
- So what you can do is override the normal program of growth (=very little), and give the whole thing a simple signal that is over 24 hours, picked up by all the transcriptional cascades and everything else, that will work over months. In our latest paper weāve shown 13 months of leg growth following one day treatment.
- Weāve looked at this in frogs and worms, in human mesenchymal stem cells, cardiomyocytes and so on, and itās very highly conserved, it works throughout the tree of life. We know itās relevant to humans because of human channelopathies. Lots of human ion channel mutations give rise to different kinds of birth defects ā even in humans these bioelectrical properties are very important for setting correct morphologies.
- What we do now in our current company (Morphoceuticals Inc.), with David Kaplan and Tufts University, is that we have bioreactors from Davidās lab that basically deliver cocktails of ion channel drugs that are picked by us to induce pro-regenerative states. We have this more or less solved in frogs and are now working on mammals and mice, hopefully someday soon in humans.
- To wrap up, the point of all of this is that we are now starting to understand the circuits to the point where we can make multi-scale models. We can start with knowing what genes are there to produce various types of ion channels, you then have to simulate the physiological tissues, and those give rise to larger organ structures and eventually you can get down to algorithmic types of control that show how the electrical activity in these tissues makes decisions about whatās going to where. So you go from genomically specified hardware, to low level and high level software that allows these cells to make the kind of decisions to robustly create and repair complex morphologies.
- In that process thereās a huge opportunity for applying machine learning, so weāve done some of this ā both to discover the electrical circuits, and to discover the modulations that are needed to use these therapeutically.
- A few examples to close up with.
- This is what a normal tadpole brain looks like, and if you perturb it with a gene mutation that changes the brain shape completely, you can create a model of the bioelectric circuit that determines the brain shape, and ask under these circumstances, what would have to change ā which channels would need to be open or closed to get back to the correct bioelectric pattern that is responsible for a normal brain. The model is now sufficiently detailed that it can tell us the channels, and based on that we can pick known ion channel drugs. And when you do this, you can get back the correct brain shape, size, function.Ā
- So this is an example of starting with basic understanding of bioelectric circuits and how they work, to a computational model that actually helps you design therapeutics to fix a rather complex issue like brain development.
- What weāre doing now is creating a complete pipeline where you start off with existing information on what ion channels are in your tissue of interest, what bioelectric state you want, and then use the model and the simulation to tell you what channel openers and blockers you need. Something like 20% of drugs are ion channels drugs, so a huge space called electroceuticals, which with the correct computational simulations you can deploy outside of their initial indications, for regenerative medicine.
- This is an example of our software you can all play with, itās online. You pick the tissue you want and it helps you to pick the correct drugs.
Iād like to thank people who have contributed to all this work, our funders, and you for listening.
Ā
Q&A
Whatās the best modern review or textbook that introduces all those people who donāt know anything about these bioelectric networks to them?
- Iāve written lots, a couple of other people have written some good ones, anybody whoās interested please email me.
Within a cell, what is the pathway for these bioelectrics to actually control gene expression? What goes on in the cell that way, how does it work epigenetically?
- On the single cell level, we know there are about half a dozen ways in which volt voltage change regulates downstream gene expression. This includes things familiar from neuroscience like voltage-gated calcium signals followed by calcium transduction, or control of transmitter movement like serotonin between cells through gap junctions or through the serotonin transporter, and this includes also some more exotic things like voltage-sensitive phosphatases, voltage-gated butyrate transporters that then hit HDAC and those kind of chromatin modification pathways, all of that is known. Itās highly unsatisfying though, because itās all single cell level data. And if you want to know why your hand doesnāt look like a foot, you still have to ask about global dynamics that determine the size and the shape and the scale, itās not enough to know what the transduction looks like on the single cell level.
In non-regenerative species after development, what do these bioelectric networks do with anything?
- I think what itās doing in adults is morphostasis ā itās keeping you together against individual cell senescence and turnover. And by the way the interface between bioelectricity and aging is not well understood at all, no one has studied it, we havenāt even touched it. But probably what it is doing is just cancer suppression and morphostasis and keeping your tissues together ā itās really activating those very dynamic morphogenic rearrangement modules that are needed to really sort of rejuvenate and restore after injury.
What controls the appearance of those electrical zones? What determines their dispersal?
- Thereās two important pieces to this. One is what underlies the hardware itself ā so you have to ask ā in order to have any kind of electrical states, you have to have the correct ion channels capable of producing them. So you need to understand what channels you have there and what their properties are. For example they might themselves be voltage sensitive, or ph gated, or whatever. And the properties of these channels are the excitable medium, on which these electrical phenomena propagate.
- Now the specific patterns, letās say that electric face pattern, itās an emergent pattern in the same way that Touring patterns emerge from an excitable chemical medium. The way I visualize this for students is that you have a collection of electric parts that make a calculator ā when you turn it on, it has a default behavior. The parts were chosen so that when the juice is turned on and the thing is running ,it will by default start off at a consistent baseline emergent state which is zero. Now past that, it has interesting properties of reprogrammability and all kinds of other things it can do, but thereās this basic state ā the parts that are shaped by evolution, this emergent pattern, and we can study that symmetry breaking. So itās a process of symmetry breaking and amplification of long-range inhibition, short-range activation, that sets up exactly two eye spots at a particular distance apart. And all of these things can be modeled.
If you chop planaria, you mentioned they have learned behaviors ā I was curious if the worms are chopped into multiple pieces, once those pieces grow if all the pieces retain those learned behaviors of the original worm?
- It was addressed first in the 60s by a guy named McConnel, and he published his observations that in fact they do retain the learned behavior. You can take a worm, train it on a particular task, chop off the tail, and raise a new worm from that tail. In 2013 we reproduced his work and itās true, they do. So what thatās telling you is that even learned behavior, not just morphological information, is stored outside the brain in some form, and is able to be imprinted on the new brain as it forms. Itās pretty remarkable, because that tail doesnāt do anything until the new brain forms, right. So it grows a new brain, and then the information is somehow imprinted. So what youāre seeing is learned information moving within the body ā super interesting, not well understood at all.
Which intervention tools are available right now mainly for regenerative medicine purposes? Is it maybe ion channel genes, or something else also available?
- The best tools as I see it right now are the ion channel drugs. In model systems at the bench, ion channel genes are useful, because genetic technologies give you the cleanest pathway information, but I think in medical application you donāt want to have to do gene therapy every time you want to target these pathways. And you donāt have to. Weāve had great success using the drugs together with a computational model ā thatās the key, you need both. Arsenal of available ion channel drugs and the model to tell you which channels to turn on and off and when to get the correct outcome.
Is there a relationship between endogenous peptide based ionophores and the ionophores youāre using?
- The vast majority of our work is not with ionophores, weāve done a little bit with ionophores. The main ionophore weāve used is monensin, but Iām sure there are others, I just donāt know about the peptide based ones. Ionophores are really kind of a sledgehammer in many ways ā we tend to use very specific ion channel drugs that hit classes of different kinds of channels.
What is the cocktail that includes DPCA?
- Thereās a variety of cocktails, the earliest was extremely simple ā it was just monense. We had one after that, which had progesterone and things like that. We are playing with DPCA. The current cocktail has five different ingredients ā stay tuned for a paper that should be published in a matter of weeks, so youāll see the cocktail and all the dosages soon.
I was impressed with your in vivo results with frog limb regeneration. Regarding in vitro application, can your drug cocktails or similar interventions be used for suspension culture to grow some vascularized organoids, or vascularized muscle tissues for clean meat application, or for in vitro you have to do something different?
- I think they absolutely can be used that way. Weāve not done that particular thing yet, but what weāve done with David Kaplanās group and some others is in vitro with cell culture. Mammalian human cell culture absolutely worked. You can keep stem cells more stem-like, you can force stem cells to differentiate, you can do those kinds of things ā exactly the same methods. I think they would absolutely work in organoids. We are currently using them in our synthetic biobots, which are basically organois but one step further ā with behavior, they move around. But itās an open area of application and nobody has gotten to it yet.
Based on your understanding of the regeneration process in your systems, what do you think would be a biological age of the regenerated tissues? Would it be younger, or the same age as the rest of the animal, or would it be older?
- I donāt know the answer to that, we have been working with Steve Horvath ā he is making a methylation clock for various organisms, and weāre waiting until he calibrates the frog one. Then we can see, that would be the easiest way to do it right now. If you have some other marker, Iād love to hear about it. Once we get the mice working, then we can easily check, that should be much easier.
You mentioned that in deer, antlers grow at rates of centimeters per day. You mentioned that in the flatworms, you were able to activate processes that are of other species. What Iām wondering is if you think it would be possible to use bioelectrical signaling to trigger bone regrowth in that same order and in the same way.
- Optimistically I think so, itās possible that weāll run into some weird aspect of deer physiology that permits it and for humans it wouldnāt work, but I donāt think so. I think these are all very fundamental things. I can tell you some speculative stories about why humans normally donāt do this kind of stuff, but I think itās there to be unlocked, I doubt itās gonna be a deer specific issue.
Have you tried setting a plate of cells atop a conductive plate with a certain pattern to see if you could guide itās development?
- Generally speaking, using external electrical signals ā electrodes basically ā you can do certain things that way. One thing you can do is to provide a vector for cell migration, thatās an easy way if you have migratory cell types, itās a great way to get them all moving in one particular direction. Whatās very hard using external electrodes is to set up a standing pattern of voltages across tissue, which is what the encoding really is. The bioelectrical code is really a pattern of voltage differences across a field of cells. Thatās actually very difficult to do with electrodes. But if you want to move cells around, that you can do with external current.
So you need to go small, like magnetic nanoparticles, or something pulling around to produce something more interesting, itās mesoscopic at that point.
- Yeah, but the tools are already here, if you want to set up a pattern that encodes the correct size and shape of the brain, which is not a simple pattern, we can already do that with drugs. And if you want a more complex pattern, I think what you would do is optogenetics, you would lay down a light mask that has the exact shape you want. Weāve done that for kick-starting tail regeneration. Right now thatās the easier path.
Is there anything that you think, considering your computer science background, could be brought from adjacent fields like microscopy or NGS sequencing, to enrich this toolkit that you mentioned? To get access to more of the morphospace?
- Absolutely, for the microscopy part ā better dyes and better imaging of bioelectric states under various conditions is really important. Nobody has done the basic profiling, Like physiomic profiling of asking every normal organ in its normal structure, what is the bioelectric map there. Weāve done that in very specific cases for some frogs and worms and things like that, but in general that data is extremely sparse. Lots more imaging, using better dyes that need to be developed, getting those physiomic datasets to complement the transcriptomic and the genomic data sets is critical.
- Regarding NGS, I feel like we already have most of the NGS that we need, because for almost any tissue we want to know what channels are there, because those are our control knobs, and for pretty much any tissue you can go online and download somebodyās profiling and you can figure out what channels are there. So thatās probably not the right limiting step, but microscopy for sure.
Outside of the translational aspect of getting things to regenerate or fixing problems in the process, how many labs in the world are studying the basic science about this? Like the aging aspect as you mentioned for example ā what changes about the nine to eleven year olds that makes their fingers not regenerate anymore, whatās involved in human liver regeneration? Are you doing anything to encourage research labs that focus on aging to nudge in this direction?
- Overall there are definitely not that many labs. Itās still relatively speaking a small field. There are people who work in bioelectricity and donāt know they work in bioelectricity. For example if you study Anderson Tawil syndrome, and youāre a geneticist and you donāt care about bioelectricity, and eventually you sequence and find out that whoa ā itās a potassium channel. Why do my potassium channel patients have craniofacial dysmorphias? Well, now youāre working in developmental bioelectricity right. So there are some folks sort of get dragged into it that way, but labs that really focus on this? There are really not that many.
- And in particular, getting money for profiling is almost impossible. From standard sources ā NIH, etc. ā youāre lucky if you can get funding for function data, like if youāre going to figure out what these ion channels do in drosophila development or something like that. You can do that, but getting money for a comprehensive profiling project like the projects figuring our what every cell in the brain is expressing (The Allen Brain Atlas)? Getting funding for that is almost impossible, so no one has done it.
What can this group do for you?
- I would love to get into aging and collaborate with anybody in this community. Generally what weāve been doing for years now to try to lower the barrier of entry for other people, is that we publish a lot of reviews and how-to protocols. We put everything online, so if you want to get into this from whatever system youāre interested in, we have literally guides with step A, B, C and you do next in each steps. We send gift baskets of reagents, we provide all the plasmids, the dye protocols, data interpretation stuff ā all of that is out there. So anybody who is interested, I would be more than happy to help you guys do this.
- Weāre certainly happy to hear from investors, anybody that wants to collaborate, drop me an email with what you do and weāll see what makes sense. Our lab is very collaborative, we work with all kinds of people, so Iād love to hear from this community.
You also mentioned you have a company, what is happening there, what are your next steps?
- The initial focus of the company is quite narrow ā limb regeneration. Weāre testing our cocktails in mice, using David Kaplanās wearable bioreactor, short-term application of our ion channel cocktail ā can we trigger limb regeneration. Thatās the beginning. Obviously my dream is to grow this into a fundamental approach for picking electroceuticals for all sorts of indications from birth defects to regenerative medicine to aging to cancer. But right now itās about limb regeneration.
What is your challenge for the longevity field?
- What I think would make a real breakthrough and move the field forward in a watershed kind of way, would be imaging these bioelectric states in all sorts of important conditions. We focus on functional experiments, because they are instructive ā we can show that when you alter the pattern, the gene expression changes, and the anatomy changes. But it would certainly make it much easier to develop those kinds of functional approaches if we had the baseline data from other model systems, various disease conditions, various human organs, this would be a real gamechanger.
Seminar summary byĀ Bolek Kerous.