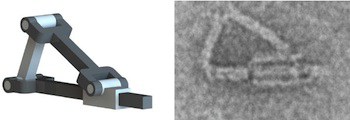
Progress in structural DNA nanotechnology seems to be accelerating. For example, a few weeks ago we cited work in which swarms of DNA nanorobots executed complex tasks in living animals. For the most part, this progress has centered on static structures, or on structures embodying small movements along loosely constrained paths. Now a team of researchers is beginning to use DNA nanotechnology to fabricate parts for machine designs based on the way macroscopic machines work by implementing well-defined motions. A hat tip to Phys.org for reprinting this Ohio State University news release written by Pam Frost Gorder “DNA Origami Could Lead to Nano ‘Transformers’ for Biomedical Applications“
Tiny hinges and pistons hint at possible complexity of future nano-robots
If the new nano-machines built at The Ohio State University look familiar, it’s because they were designed with full-size mechanical parts such as hinges and pistons in mind.
The project is the first to prove that the same basic design principles that apply to typical full-size machine parts can also be applied to DNA—and can produce complex, controllable components for future nano-robots.
In a paper published this week in the Proceedings of the National Academy of Sciences [abstract], Ohio State mechanical engineers describe how they used a combination of natural and synthetic DNA in a process called “DNA origami” to build machines that can perform tasks repeatedly.
“Nature has produced incredibly complex molecular machines at the nanoscale, and a major goal of bio-nanotechnology is to reproduce their function synthetically,” said project leader Carlos Castro, assistant professor of mechanical and aerospace engineering. “Where most research groups approach this problem from a biomimetic standpoint—mimicking the structure of a biological system—we decided to tap into the well-established field of macroscopic machine design for inspiration.”
“In essence, we are using a bio-molecular system to mimic large-scale engineering systems to achieve the same goal of developing molecular machines,” he said.
Ultimately, the technology could create complex nano-robots to deliver medicine inside the body or perform nanoscale biological measurements, among many other applications. Like the fictional “Transformers,” a DNA origami machine could change shape for different tasks.
“I’m pretty excited by this idea,” Castro said. “I do think we can ultimately build something like a Transformer system, though maybe not quite like in the movies. I think of it more as a nano-machine that can detect signals such as the binding of a biomolecule, process information based on those signals, and then respond accordingly—maybe by generating a force or changing shape.”
The DNA origami method for making nano-structures has been widely used since 2006, and is now a standard procedure for many labs that are developing future drug delivery systems and electronics. It involves taking long strands of DNA and coaxing them to fold into different shapes, then securing certain parts together with “staples” made from shorter DNA strands. The resulting structure is stable enough to perform a basic task, such as carrying a small amount of medicine inside a container-like DNA structure and opening the container to release it.
To create more complex nano-machines that could perform such tasks repeatedly, Castro joined with Haijun Su, also an assistant professor of mechanical and aerospace engineering at Ohio State. Combined, the two research teams have expertise in nanotechnology, biomechanics, machine engineering and robotics.
Castro said there are two keys to their unique approach for designing and controlling the machines’ motion. The first involves making certain parts of the structure flexible. They make flexible parts from single-stranded DNA, and stiffer parts from double-stranded DNA.
The second key involves “tuning” the DNA structures so that the machines’ movements are reversible and repeatable. The researchers dot their structures with synthetic DNA strands that hang off the edges like the awning of a roof. Rather than join portions of the machine together permanently, these strands are designed to act like strips of hook and loop fasteners—they stick together or unstick depending on chemical cues from the machine’s surroundings.
In the lab, doctoral students Alexander Marras and Lifeng Zhou took long strands of DNA from a bacteriophage—a virus that infects bacteria and is harmless to humans—and “stapled” them together with short strands of synthetic DNA.
First, they joined two stiff DNA “planks” with flexible staples along one edge to create a simple hinge. Castro likened the process to “connecting two wooden 2×4’s with very short pieces of string along the 4-inch edge at one end.”
They also built a system that moved a piston inside a cylinder. That machine used five planks, three hinges and two tubes of different diameters—all made from pieces of double-stranded and single-stranded DNA.
To test whether the machines were moving properly, they imaged them with transmission electron microscopy. They also labeled the DNA with fluorescent tags, so that they could observe the shape changes with a spectrofluorometer. Tests confirmed that the hinges opened and closed and the piston moved back and forth—and that researchers could control the motion with the addition of chemical cues to the solution, such as additional strands of DNA.
This approach of designing simple joints and connecting them together to make more complex working systems is common in macroscopic machine design, but this is the first time it’s been done with DNA—and the first time anyone has tuned the DNA to produce reversible actuation of a complex mechanism.
The research team is now working to expand the design of mechanisms for tuning the machines, and they will also attempt to scale up production of the machines for further development.
This paper introduces a methodical strategy to bringing precise control of complex motions to mechanical devices fabricated using structural DNA nanotechnology, and in particular, the scaffolded DNA origami method. They integrate relatively stiff double-stranded DNA and flexible single-stranded DNA links with stiff origami-based nanostructures “to create mechanical devices capable of precise motion over ~10-100-nm range.” They combine these with stiff origami components integrated using complementary geometries, such as concentric structures designed for one to slide within the other. Structures were verified by transmission electron microscopy, and energy landscapes calculated from the distribution of shapes seen.
Beginning with simple slider and hinge joints, they demonstrate the ability to design constrained linear and rotational motions with tunable flexibility. Combining these simplest components they designed two mechanisms that integrate several joints to achieve 2D and 3D complex motions. A crank-slider combined three hinges and one slider joint. The second mechanism combined four hinges and four square origami components to form a “Bennett linkage” that can alternate between a compact and an expanded conformation. Because the compacted configuration of the Bennett linkage is a higher energy state, the researchers were able to demonstrate distributed actuation by using a series of strand displacements to program reversible conformational changes, first storing mechanical energy and then performing mechanical work.
With the eventual accumulation of actual nanoscale parts fabricated using structural DNA nanotechnology, it will be possible to ask if these parts are precise enough and stiff enough to use to assemble complex microscale DNA machine systems. Could such parts transmit mechanical force and control complex motions deterministically, as is done with conventional macroscopic machinery? Could such systems muster forces sufficient to make and break strong covalent bonds, as is envisioned in theoretical proposals for atomically precise manufacturing of diamondoid machinery? What enhancements could be achieved by introducing other components, such as proteins or other catalysts into DNA molecular machinery? Or could they be used as intermediates to build systems of somewhat stiffer components from somewhat stiffer materials, as an instance of using crude tools to build more precise tools? Some critics of proposals for high throughput atomically precise manufacturing (APM) have argued that designs for macroscale mechanical systems do not scale well to the nanoscale, so that entirely new designs based more on evolved biological machines will be necessary to work on the nanoscale. The above research may be a step toward evaluating such critiques.
—James Lewis, PhD